“Stopping climate change” is the wrong goal
Humanity needs to create climate control.
The following is Chapter 5, Section 3, from the book The Techno-Humanist Manifesto by Jason Crawford, Founder of the Roots of Progress Institute. The entirety of the book will be published on Freethink, one week at a time. For more from Jason, subscribe to his Substack above.
Chapter 5, Section 3: Solutionism
Progress solves problems, but creates new ones. I have claimed that the new ones are better problems to have, that they can be solved in turn by more progress, and that history shows a pattern of us doing so.
In many people’s minds, however, there is a major, theory-exploding counterexample: a problem massive in scope, global in reach, and generational in timescale; one that is inherently caused by industrial activity; one that we have known about for decades but not taken nearly sufficient action on; one that threatens us and future generations, with no solution in sight. That problem is climate change.
In a common narrative, climate change is an existential threat to humanity, and drastic measures are needed to stop it, up to and including massively slowing down or even reversing economic growth.1 In response, some complacent optimists ignore the threat or decide that it isn’t real.2
As a solutionist, I am eager to embrace the reality of the problem. But “stopping climate change” is the wrong goal. It is an anti-human, anti-agency framing, focused on negating the impacts of human activity. The techno-humanist framing is that humanity should create climate control.
The case for climate control
The climate matters.3 It affects crops and livestock, travel and shipping, coastal real estate, the spread of disease, and human comfort. The costs of unmitigated climate change are hard to estimate, but even the more optimistic models expect that 4º C of warming would cause world GDP in 2100 to be 1–8% lower than in a world with no warming (and the more pessimistic models estimate 10–23%).4 This is not an existential threat, but it is a problem measured in the trillions or tens of trillions of dollars per year.
Let me state a general principle:
Anything that matters to humans should be under our control.
The climate matters—so we should control the climate.
If you are optimistic that the impacts of climate change will be mild and manageable, and therefore less worried, note that these damage estimates are based on the current, relatively modest rate of growth in energy usage. But ideally, we would massively increase energy usage. If we 10x world energy production, it seems much more likely that we will also need to mitigate any effects on climate.
Further, the impacts of climate change are highly uncertain, as they are difficult to model. Not only are there wide error bars on our median estimates, there is also a small chance of hitting extreme scenarios, such as crossing “tipping points” past which it would be very hard to reverse the effects. The more the effects are uncertain, the more we should be in control of the causes.
Indeed, given this uncertainty and the complex nature of the system, it is not enough to aim for a static state that we can specify today, armed with only our current, relatively meager knowledge—a goal such as “net zero” or “350 ppm” or “limit warming to 2º C.” Instead, we need to build a dynamic system of monitoring and control that lets us adapt in real time to changing circumstances and new information.
Climate has never been under human control. Instead, we insulate ourselves from it in sealed boxes, we harden our infrastructure against it, we irrigate our fields and adapt our crops. We should be more ambitious. We should have a climate-controlled planet just as today we have climate-controlled rooms.
In this pursuit, we have two factors on our side. One is that climate change is a slow-moving and relatively predictable problem—the best kind to have. It unfolds over decades; some effects, such as ice sheet “collapse” and sea level rise, unfold over centuries.5 The other is that there are an array of technological approaches to the problem, which have gone scandalously under-studied by experts and under-reported in popular media. I sketch them out below.
Every solution creates new problems, and solving any problem of control creates a new problem of governance. If we install a thermostat on the Earth, what should we set it to—and how could we ever agree? Regional communities have dealt with such challenges before, such as the governance of water systems,6 but this would be the first one at planetary scale. I’m not going to solve those political problems in this essay. I’m also not going to address exactly how fast we should build a climate control system, or how much we should be willing to spend on it; Nobel-laureate economists who have been modeling this for decades do not agree. My purpose here is only to show that we can solve the problems of climate change by moving forward with technology and industry—not by rolling them back.
How, exactly?
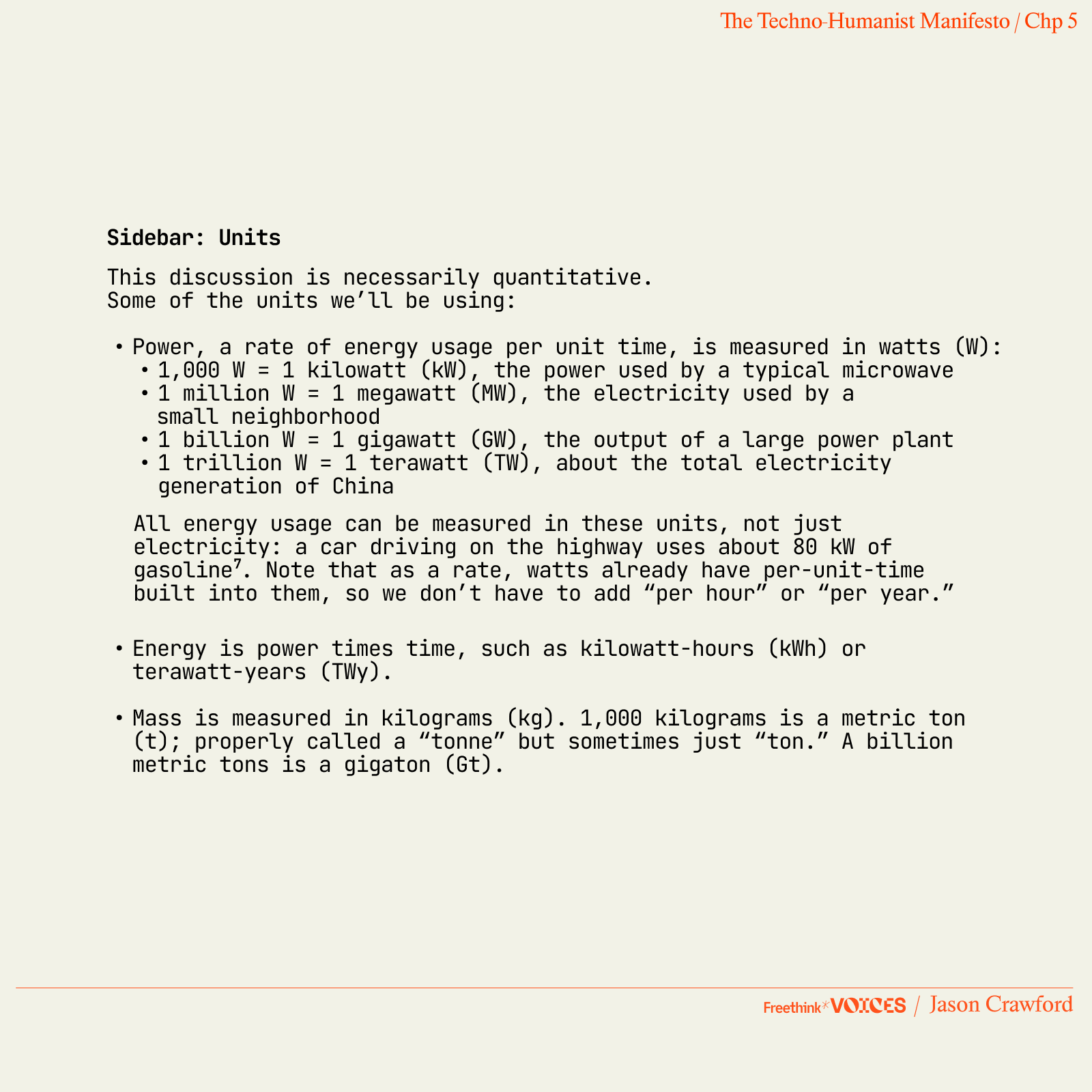
Planetary accounting
Warming or cooling the planet is a matter of managing its energy balance.
The Earth receives about 173,000 TW of light energy from the Sun. Roughly 30% of that is immediately reflected back into space (mostly as visible light), leaving about 122,000 TW that is absorbed and becomes heat. The vast majority of that heat energy is radiated away into space (mostly in the infrared spectrum), but about 300 TW remains and warms the planet.8
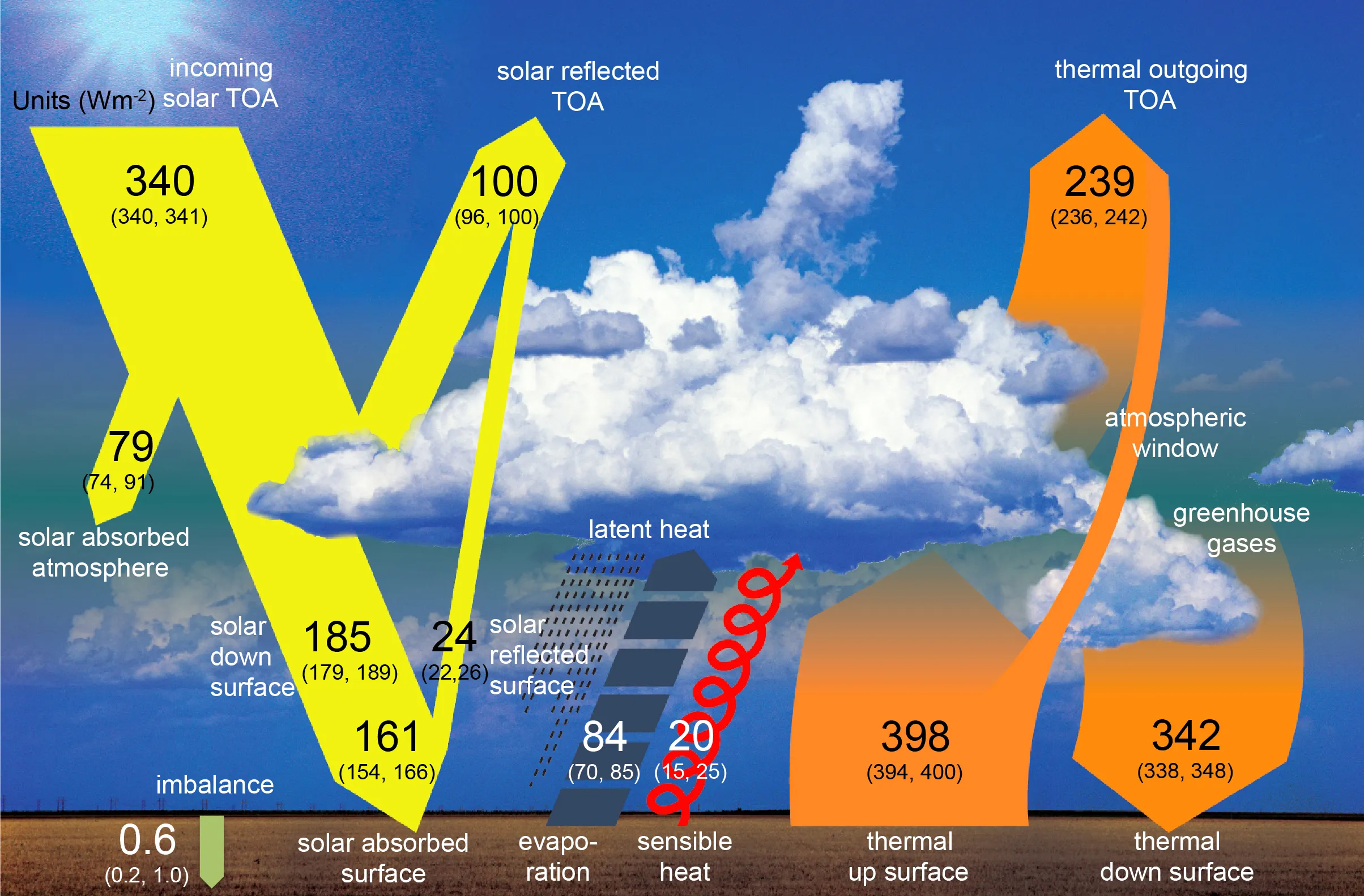
The amount of heat energy that is radiated away, labeled “thermal outgoing” in the diagram above, depends on greenhouse gases (GHG), mostly CO2 and methane.9 These gases are transparent to solar radiation, which is in the visible spectrum, but absorb thermal radiation, which is infrared. Hence they act like a one-way door for energy, letting it in but then trapping it here.
In the first decade of this century, natural processes put 727 Gt/yr of CO2 into the atmosphere; another 33 Gt/yr, or just 4.3% of the total, came directly from human activity (mostly fossil fuel use, cement production, and deforestation). 746 Gt/yr of that was removed from the atmosphere, mostly by plants and the ocean, leaving 14 Gt/yr that remained in the atmosphere, adding to the total.10 (The figures today are a bit higher, but the overall picture is the same.)
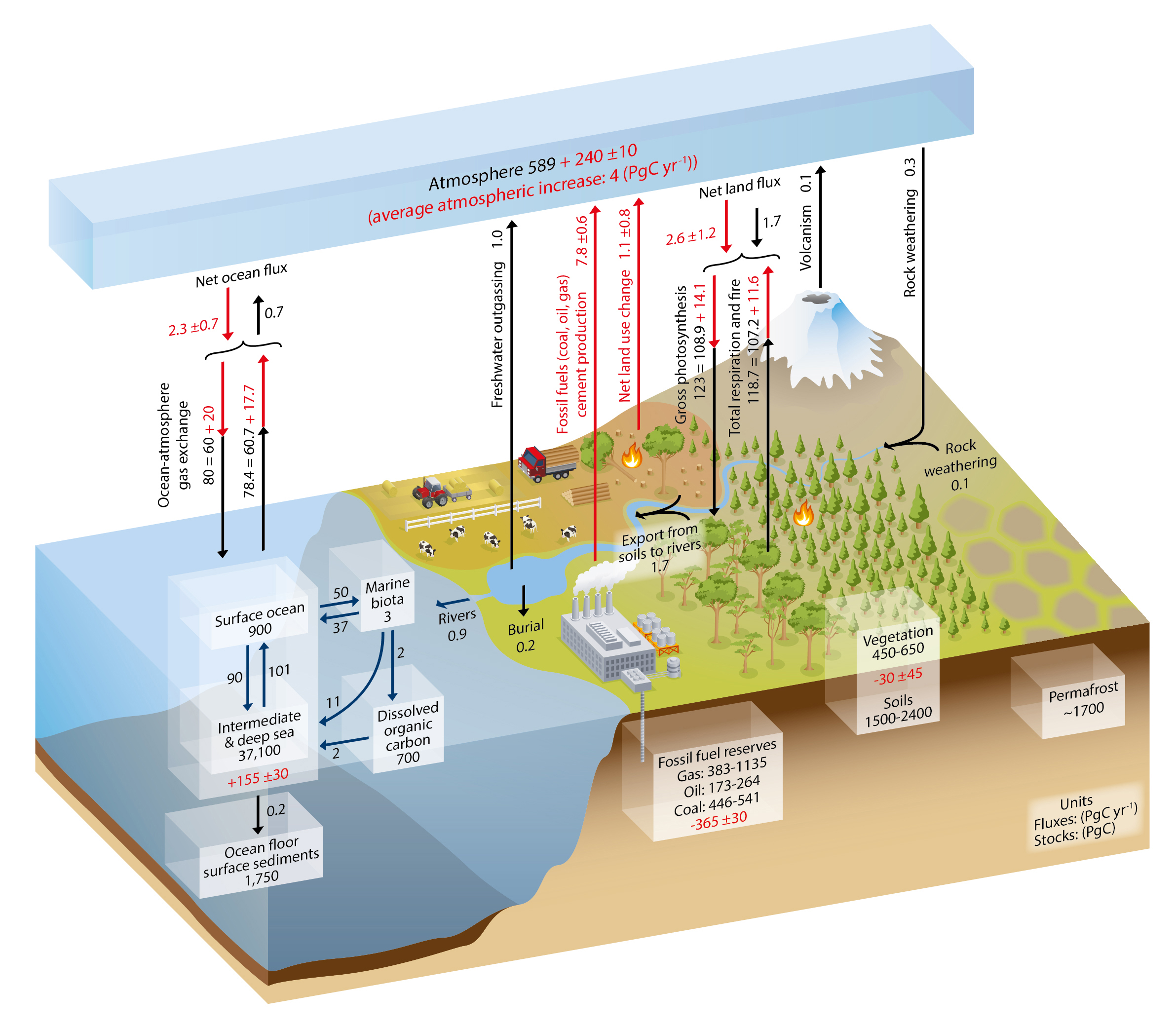
Note that in both the case of energy and the case of CO2, the inflows and outflows are both quite large, and the net flow is small compared to them: the CO2 imbalance is less than 2% of the CO2 inflow, and the energy imbalance is less than 0.2% of the energy inflow. That is, to make a large change in the net flow, the impact required is small compared to the total flow in either direction. That’s part of why this is tractable.
So, to control the rate of warming or cooling, and thus to control the temperature of the Earth, we need to control these flows: balancing them when we want to stabilize the temperature; driving them positive or negative when we want to change it. Our levers to do this are:
- The amount of solar energy immediately reflected back into space, vs. absorbed by the planet (“solar reflected” in the diagram above). This depends on the reflectivity of the Earth, known as albedo.
- The amount of energy released (as infrared radiation, “thermal outgoing” in the diagram). This is mainly determined by greenhouse gases, so we actually have two levers here:
- The amount of GHGs added to the atmosphere, and
- The amount of GHGs removed.
We lack climate control right now because, first, our infrastructure inadvertently emits a lot of GHGs, and we have no realistic way of significantly dialing that down; and second, because we have no infrastructure for GHG removal or for albedo control. So let’s look at each in turn to sketch a picture of a climate control infrastructure.
Emissions
Here’s where GHG emissions come from:11
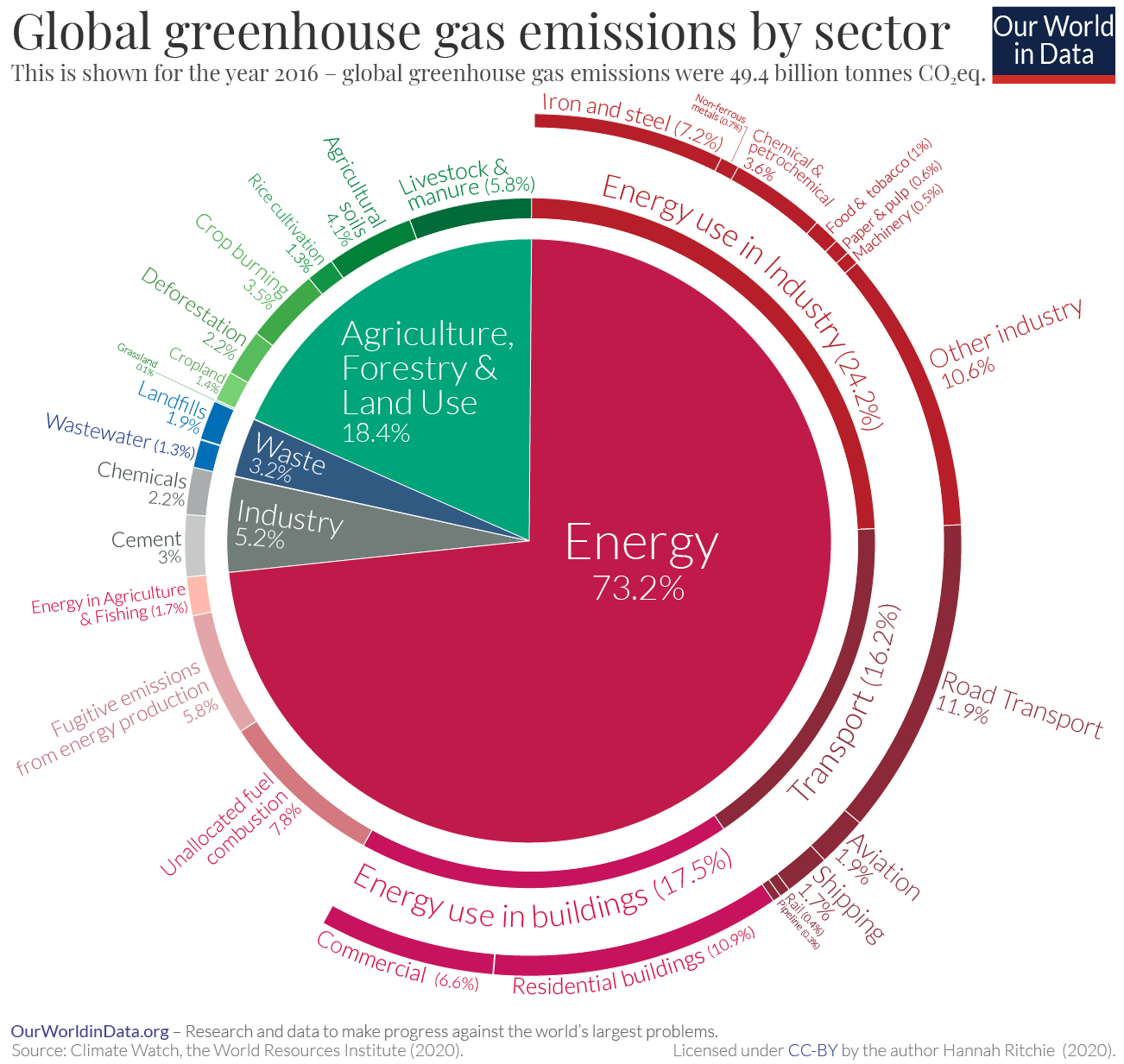
Almost three-quarters of emissions are from energy usage, so that’s what we’ll focus on here.
Most of those emissions are CO2 from coal, oil, and gas.12 Our lives and our world depend on these precious fuels; our civilization was built on them. But by depending on them, we are locked into a certain minimum amount of emissions. To achieve climate control, we need to lower this floor.
From a techno-humanist perspective, though, energy usage is non-negotiable. Reducing energy usage, or even slowing the rate of growth of energy usage—by increasing the price of energy, or making it less reliable—would quickly harm quality of life far more than a warmer climate.
One way to reduce emissions is to capture them at the source, using chemical methods. This technology exists for CO2 emitted by industrial facilities such as power plants, and it can reduce emissions by more than 90%.13 However, it costs around $50–100/t of CO2, plus maybe $10/t for transportation and storage.14 At a total cost of, say, $80/t, that would add about $0.03/kWh to the cost of electricity in the US,15 more than a 20% increase.16 So let’s consider zero-carbon energy sources, to see if they can meet our needs.
We need energy that is cheap, abundant, and reliable.17 Our energy system must provide terawatts of power, for pennies per kWh, 24/7/365. Where can it come from?
Here’s the theoretical maximum energy available from various sources, divided into those best considered as renewable flows, vs. fixed stocks that we draw down.18 For context, total world energy usage today (not just electricity, but all forms of energy) is roughly 20 TW:
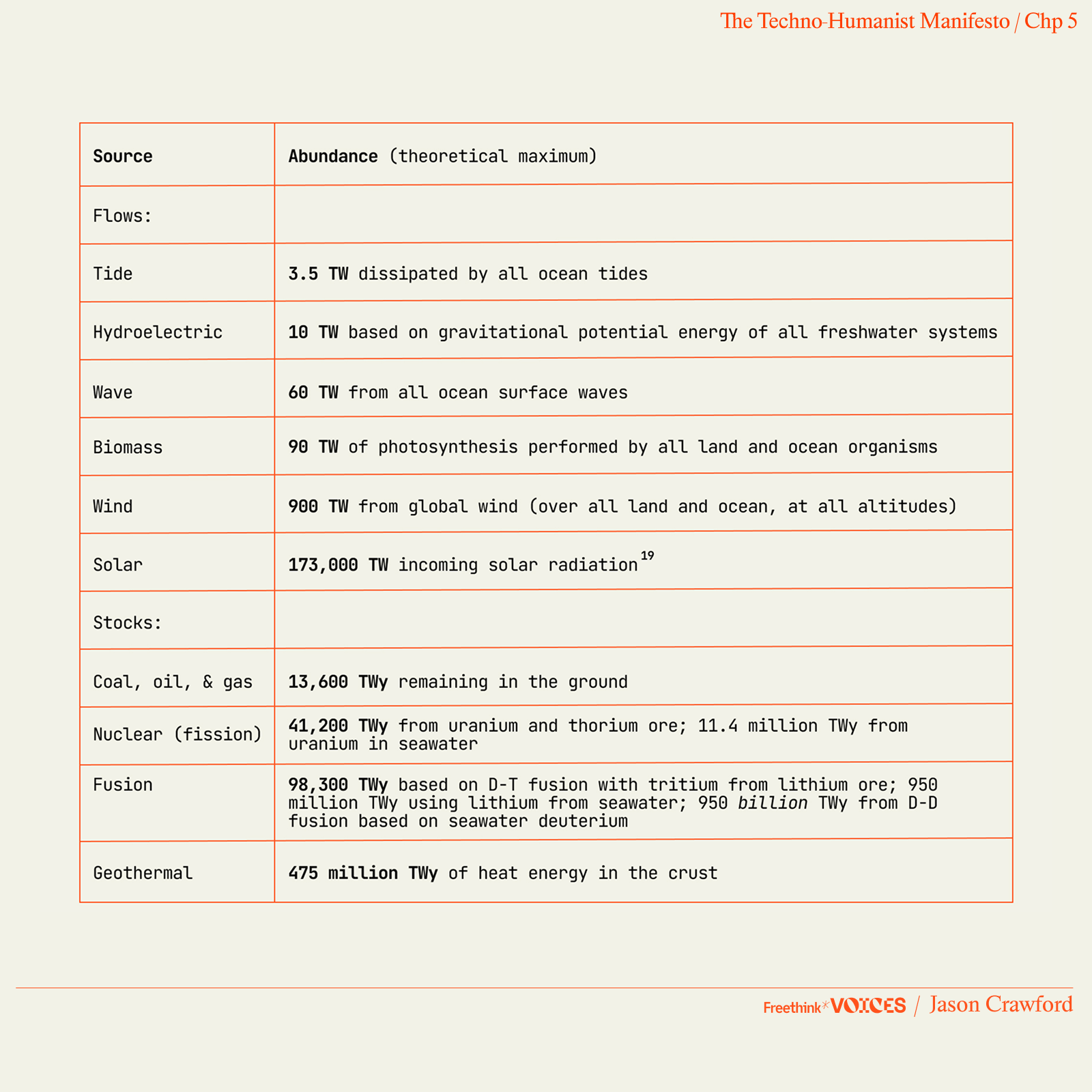
The practically extractable energy from each source is much less than the theoretical maximum: to use all biomass, we’d have to burn all new plant growth on the planet for fuel; to use all wind energy, we’d have to put windmills all over the land and ocean, and also somehow suspend them in the atmosphere; to use all solar energy, we would have to literally blot out the sun. But these estimates are useful as an upper bound that doesn’t depend on any assumptions about technology, economics, or politics; and the differences in order of magnitude between them are instructive.
At the margin, all economical sources are worth using. But to architect a global system, we should focus on energy sources with massive scale. Below, I’ll consider the flows that can provide at least 50x world energy usage, and the stocks that contain at least 2000x (an admittedly arbitrary cutoff).
Solar is currently the cheapest of these—at least, when sunlight is available. Solar panels now cost around $0.30/W (and the cheapest ones are under $0.10/W), and a full utility-scale solar installation is ~$1/W or less, leading to an electricity cost of around $0.044/kWh. And it is getting rapidly cheaper: prices have fallen 90% since 2010, thanks to process improvements and economies of scale.20 Solar panels use a cheap, abundant material: silicon is the second most common element in the Earth’s crust. We already have a lot of industrial experience working with silicon, and infrastructure to process it. Solar panels can be mass-manufactured in factories, they fit in shipping containers for distribution, and they’re relatively easy to install. Because of this, installations are growing rapidly, with solar power generation roughly doubling every 3 years since 2014.21
But solar is intermittent, owing to daylight hours and cloud cover: the actual power it generates is around 25% of its full capacity in the US, and less than 20% worldwide.22 And providing battery backup adds to the cost—by an amount that rises steeply as you approach 100% reliability. One recent model concluded that, even in a reasonably sunny location like Atlanta, a solar-plus-battery system that provided 80% of electricity demand would cost over 3x a system that used solar alone.23 To provide 99% of electricity demand, the cost of electricity would rise to about 7x—and even 99% is well below the availability of the grid, which is over 99.9% in the US and as much as 99.999% in places like Singapore.24 Costs will improve, as the cost of battery storage is dropping about as fast as the cost of solar.25 But for the foreseeable future, solar can be at most one component of the power grid, complemented with dispatchable power; or it can be applied to local industrial uses that can operate less than 100% of the time.26
Nuclear (fission) is the inverse of solar: it provides constant, reliable power, but it is expensive—at least in the West today. One estimate puts the cost of electricity from the recently completed Vogtle 3 and 4 plants at $0.19/kWh, more than 4x the cost of (intermittent) solar.27
Nuclear’s advantages come from the mind-boggling energy density of its fuel, which contains more than a million times as much energy per kilogram as hydrocarbons: one gummy-bear sized pellet of uranium contains more energy than a ton of coal.28 This is why there are millions of terawatt-years of fuel reserves: a very small mass of uranium or thorium lasts a very long time. “Refueling” a nuclear power plant is approximately an annual maintenance task, like changing a battery. The fuel cost of a nuclear power plant is a very small portion of the total cost of electricity, contributing less than a penny per kWh29 (and would be even smaller in advanced nuclear reactors that make much more efficient use of fuel).
Nuclear is expensive because it’s slow and expensive to construct and license a nuclear plant in most places today, and that financing can only be repaid with expensive energy. Capital costs thus make up more than 80% of nuclear electricity costs.30 But there is no fundamental reason in physics or engineering why nuclear plants can’t be built much faster and at much lower cost.31 While nuclear construction in the US and UK costs around $10/W; in Korea, India, China and Japan it costs $2–3/W.32 High costs are the product of an industry that never drove down the learning curve to optimize its processes, and a regulator that explicitly refuses to perform cost-benefit calculations.33 Fortunately, there is a new generation of nuclear founders determined to work around or ram through these barriers. A few I am keeping my eye on include Last Energy, Antares, Oklo, and Valar Atomics.
There is also hope from an entirely different form of nuclear: fusion. Fusion fuel, amazingly, is even more energy-dense than fission fuel; and it has the potential to be even more abundant. But so far, fusion is still a research project. Since the mid-20th century, it’s been perpetually a decade or more away; one of those technologies about which people joke: “it’s the future—and it always will be.” But now it is less than a decade away, according to two fusion startups: in 2023, Helion Energy announced a plan to have a 50 MW plant online by 2028; in 2024, Commonwealth Fusion Systems announced a 400 MW plant that would “deliver power to the grid in the early 2030s.”34 Many observers are skeptical, but given two independent announcements, I am optimistic. We’ll know soon. Helion is aiming for long-term electricity costs of $0.01/kWh.35
So: solar is cheap but intermittent, fission is reliable but (currently) expensive, and fusion depends on technologies still in development. The ideal source would provide reliable power 24/7, cheaply, using only relatively established, “boring” technologies.
Enter geothermal power.36 Geothermal, using heat from the Earth, is reliable and relatively cheap ($0.064–$0.106/kWh, according to one estimate37). Until recently, it has not been abundant, because it could only be accessed in the few spots where underground heat comes to the surface, such as volcanic islands. But geothermal energy is everywhere, provided you can drill deep enough—which we now have the technology for, thanks to the oil & gas industry. Fervo Energy, for example, is building a 400 MW geothermal plant in Utah that is slated to be online in 2026 and fully ramped up by 2028.38
So far, we’ve been discussing electricity generation. But electricity is only about 20% of world energy usage.39 Most of the rest comes from directly burning fossil fuels to power vehicles (from motorcycles to cargo ships) or to make heat (whether to cook your dinner, to smelt iron, or anything in between).
Some of this infrastructure can be electrified, such as cars and building heat. Light-duty vehicles and commercial and residential buildings together represent about 30% of GHG emissions in the US.40 But not everything can be easily electrified. Airplanes, for instance, will run on hydrocarbon fuels for the foreseeable future: the power-to-weight ratio is crucial in aviation, and jet fuel holds 50 times more energy per kilogram than batteries.41
Instead of electrifying infrastructure that runs on hydrocarbon fuels, another approach is to continue using fuels—not taken out of the ground, but synthesized from CO2 taken out of the atmosphere. These fuels will release CO2 when burned, but since they are created by removing CO2, their net impact is zero: this amounts to cycling carbon in and out of the atmosphere.
This process is energy-intensive, but if powered by sufficiently cheap, abundant energy, it can produce affordable fuels. Terraform Industries, for instance, is using solar power to synthesize methane, the main component of natural gas, from atmospheric CO2. Using solar for fuel synthesis also obviates the problem of intermittency: production simply happens during daylight hours, and the equipment can be made cheap enough that a low capital utilization doesn’t ruin the economics of the plant. In effect, this is replacing batteries with methane as a form of storage. Terraform is aiming for a price of $10 per 1,000 cubic feet of natural gas, which is about 2x historical US prices but which would be competitive in many international markets.42 Terraform has grand ambitions for scale: they envision a global fleet of 400 million plants in twenty years, which would produce almost 27 TW of power.43 Valar Atomics is building a similar process, but using nuclear power, and also plans to eventually be competitive with market prices for fuels.44
Hydrocarbons are an excellent form of energy! Hydrogen (that isn’t bound to oxygen) has a lot of energy potential, and carbon chains are a handy way to carry lots of them around. They are highly energy-dense, they can be transported anywhere and stored for long periods of time, and we already have a vast industrial infrastructure to transport and use them, including almost a terawatt of electricity generation from natural gas.45 The problem with fossil fuels isn’t the fuel part, it’s the fossil part.46 Contra popular visions of a fully electrified world, we can achieve a clean energy future while still burning lots of hydrocarbon fuels.
CO2 removal
It’s not enough to reduce the amount of GHGs we emit. If we want to control the climate, then we need the ability to dial GHGs up and down as we please. This requires negative as well as positive emissions.
And even if you only care about reaching net-zero emissions, this is essentially impossible without negative emissions. First, reaching net zero would take decades, and well over $200 trillion of capital investment.47 Second, not all emissions can be eliminated or even captured. There are crucial industrial processes that emit CO2 for chemical reasons, including steel and cement (which together are about 10% of GHG emissions). Another ~18% of GHGs come from agriculture, notably methane from livestock and N2O from soil fertilization. The technologies to replace those things—hydrogen-based iron smelting, synthetic meat, vertical farming—are decades away.
We need the ability to suck GHGs out of the sky. And we’ll need to do it at massive scale: just as power sources must be measured in terawatts to make a dent in world energy; carbon capture must be measured in gigatons to make a dent in world emissions. Since CO2 and methane have the biggest impact on warming, and methane turns into CO2 after about a decade, we’ll focus here on CO2 removal in particular.
There are chemical processes to remove CO2 from the air, known as direct air capture or DAC. These processes use chemicals called sorbents or solvents that bind to CO2. The CO2 must then be released from the sorbent or solvent, typically by heating, in order to re-use it to grab more CO2. These processes, then, don’t permanently sequester CO2: they merely concentrate it out of the atmosphere into an enriched stream. Then we need to store it, perhaps by injecting it deep underground, or use it as an input, such as in the fuel synthesis processes mentioned above.
The challenge with all CO2 removal is that CO2 concentrations in the atmosphere are small in relative terms: around 0.04%. This makes DAC the chemistry equivalent of looking for a needle in a haystack. Thus it is highly energy-intensive: the theoretical minimum is about 180 kWh/t, but the best processes today are far from that, 1,600–4,400 kWh/t,48 most of which is used for heat. One potential avenue to improve efficiency is using electricity rather than heat to release the CO2 from the sorbent or solvent, but this is still being researched.49
Largely because of the energy requirements, the total cost of DAC right now is $250–600/t.50 To remove all human-emitted CO2 by this method would therefore require at least several terawatts of power, and would cost some $10–20 trillion per year—a significant fraction of world energy usage and of world GDP. In other words, this is an expensive and energy-intense method of carbon removal, unless and until major efficiency improvements are achieved.
But recall that natural sources already remove well over 700 Gt/yr of CO2 from the atmosphere. We only need to enhance that activity by a few percent to control the CO2 balance. What are these natural processes?
One is photosynthesis. Plants, algae, and other organisms take in CO2 and water, produce hydrocarbons that are incorporated into their biomass, and emit O2.
About half of this is done on land, mostly by forests, which already remove a net 8.8 Gt/yr of CO2, or about 90% of the net land flux.51 One hectare of planted forest can remove about 4 to 40 t/yr of CO2, so we need something around a hundred million hectares per Gt.52 There are about 4 billion hectares of forest on the planet, so making a dent in carbon removal would require significantly expanding global forest.53 This would use an enormous amount of land: various studies have estimated 900–950 million hectares—about the size of the Sahara desert.54
It also would require an enormous amount of water, roughly 9 m3/day per hectare of land that isn’t otherwise irrigated.55 If, say, a third of those 950 million hectares have to be irrigated, that’s over 1 trillion m3/yr, a 25% increase in global fresh water consumption.56 Much of this would have to be provided by desalinating seawater, which would require around 300 GW of energy.57 This is a good application for solar power: many of the sites that need irrigation are near the equator, and as with methane synthesis, the equipment can be run intermittently, without battery backup. Desalination with cheap energy has achieved costs of around $1/m3 of water;58 and the equipment to do so can fit on less than 3% of the land it irrigates.59
The cost of forestation varies widely. One study cites a mean cost of $2,500/hectare, plus a 50-year management cost of $6/hectare/yr, or $2.4 trillion to plant 950 million hectares, followed by $5.7 billion/yr in management.60 A potential challenge is that a mature forest sequesters less carbon, so for maximal effectiveness, after 30–50 years, we’d need to harvest the wood and use it as lumber, or bury it somewhere where it won’t rot (perhaps after charring it into a high-carbon residue known as “biochar”).
The other major source of photosynthesis is ocean phytoplankton, such as cyanobacteria and green algae.61 Phytoplankton growth, like that of plants on land, is rate-limited by a few key nutrients, such as iron, nitrogen and phosphorus.62 Fertilizing the ocean with a combination of these nutrients could enhance growth. The nutrients themselves are very cheap, so this method could capture carbon at a price far lower than DAC, possibly as low as $2/t.63 It uses no land or fresh water, and no energy or machinery beyond what is needed for the fertilization. It is a controllable process: if we stop fertilizing, the plankton stop growing. And the potential scale is vast: by one estimate, less than 1% of the ocean surface could absorb more than all human-emitted CO2.64 (The ocean already holds 38,000 Gt of dissolved carbon—thousands of times current annual human emissions.)
One challenge is making sure that the phytoplankton (or whatever animals eat them) sink into the deep ocean, so that the embodied carbon is actually sequestered. Alternatively, we could attempt to grow different types of marine biomass, such as kelp, which might be easier to sink.65 This approach may also have side effects we don’t yet understand: we need more research. (It’s also controversial, and currently contravened by two different UN resolutions.66)
In the long run, it may be possible to increase the efficiency of biomass carbon removal through genetic engineering—e.g., by creating “carbon-eating” trees,67 phage-resistant cyanobacteria (which would be more likely to sink),68 or more efficient photosynthesis.
Apart from photosynthesis, CO2 is also absorbed into rock.69 Atmospheric CO2 combines with water to form carbonic acid, which reacts with minerals such as magnesium or calcium to form carbon-containing compounds. This occurs naturally via rainwater and in the ocean, but at small scale, about 1 Gt/yr of CO2.70 This rate, however, is determined not by the amount of rock, but by the amount of rock surface area exposed to water. This means we can greatly accelerate weathering by mining suitable rocks, such as olivine, and grinding them into silt. A metric ton of olivine silt, spread in shallow waters, would capture 1.25t of CO2 and sequester it permanently. (As an added benefit, it would help counteract ocean acidification, which is one of the side effects of increasing CO2 levels.)
Suitable minerals are abundant and widespread: the Samail Ophiolite, a huge geologic formation in Oman, has by itself enough material in the top 3 km to sequester 20,000 Gt of CO2—over 500x current annual human emissions. One estimate puts the total planetary capacity for mineral CO2 sequestration at 60 million Gt,71 which is orders of magnitude more carbon than in estimated remaining fossil fuel reserves—we’ll run out of fossil fuels before we run out of rock to sequester the carbon in.72
The technology for mining, crushing, and transporting rock is mature, and the infrastructure is widespread. The process has such a high return on invested energy that the mining equipment could even run on fossil fuels without significantly impacting net carbon removal efficiency. The main cost is the energy required to mill the rock into sufficiently fine particles: one analysis cites a ballpark figure of 80 kWh/t of CO2 (more than 10x as efficient as DAC today).73 Even at a modest price of $0.10/kWh, that’s only $8/t of energy costs. The cost of capital for the milling machinery would only be around $1.60/t, so the total cost could be in the ballpark of $10/t—and even lower with cheaper energy. Unlike biomass approaches, mineral capture is relatively permanent, and it has less risk of disrupting ecosystems. Altogether, this strikes me as one of the most promising approaches.
An intriguing long-term idea is to use carbon-capturing enzymes, not in living cells but in cell-free processes in bioreactors. This could be RuBisCO, the enzyme that drives photosynthesis; an enzyme that catalyzes a different carbon-capturing process; or an engineered or enhanced version of one of these. This method could have the best of the biological and chemical approaches: an energy-efficient process, possibly even one that could run on sunlight, but with the high controllability and small land footprint of a chemical plant. This technology is still in the earliest stages, however.74
If one or more of these technologies can be massively scaled up, and if they can efficiently drive down the learning curve as they scale, we could conceivably remove all excess CO2 for an annual cost in the hundreds of billions or less, that is, a small fraction of a percent of world GDP.
Albedo control
Controlling the net flow of GHGs into or out of the atmosphere is a key part of our climate control infrastructure, but it is not enough. The infrastructure described above will take decades to scale up. And even when it is in place, large changes to the GHG content of the atmosphere will take a long time: even at, say, net negative 10 Gt CO2/yr (a very ambitious target), it would still take more than 30 years to reduce overall atmospheric CO2 levels by even 10%.75 We need another lever in the system—a way to control climate on a scale of years, not decades.
This is why we also need to control the last part of the equation: the amount of sunlight reflected immediately vs. absorbed. We need a dial on the albedo of the planet, known as solar radiation management (SRM). Lowering incoming solar radiation by less than 2% would cool the planet by 2º C.76
Of the reflected radiation, only about a quarter is reflected from the Earth’s surface; the rest is reflected from the atmosphere.77 So we will focus here on increasing atmospheric albedo.
The simplest way to do this is to put aerosols into the stratosphere that will back-scatter incoming sunlight.78 This can be done with common, abundant materials, such as sulfur dioxide (SO2) or calcite (CaCO3, basically chalk). One million metric tons of SO2 would reflect 158 TW of solar energy, about half of our energy imbalance of 300 TW.79 Another way to quantify this is that one gram of SO2 in the stratosphere offsets the warming of 1 metric ton of CO2 for a year. This million-to-one ratio of effectiveness makes this approach scalable and cheap.
SO2 injection occurs naturally from volcanic eruptions: the 1991 Mt. Pinatubo eruption contained some 15–20 million tons of SO2, and lowered global temperatures by about 0.5º C for more than a year.80 We could do it artificially using planes or balloons. One startup, Make Sunsets, will launch a balloon to release SO2 in the stratosphere for you right now. Their costs are only $0.28/g,81 and even this could be driven down by orders of magnitude at scale: Casey Handmer, based on a scenario using the “largest practical single-use balloon,” estimates costs as low as $0.35 per kilogram of SO2—remember, that’s enough to counter the warming of 1,000t of CO2 for a year.82 At that price, we could reflect the entire excess heating of all human CO2 since the Industrial Revolution for a total of $634 million/yr. Round up to a cool billion for overhead, and it would still only cost one penny per month for each person on the planet—or if Americans were feeling generous, we could fund the whole thing ourselves for less than $3/person/yr. Compare that to the cost of your Spotify subscription.
SO2 is a pollutant, and contributes to acid rain.83 But it only causes those problems near the ground. When injected into the stratosphere, it will linger there for maybe two years, reflecting sunlight for us, before falling. This is much more efficient than the SO2 already emitted by industrial pollution into the lower atmosphere, which only stays there about a week.84 Because of this, the amount of stratospheric SO2 needed to offset global warming is only a few percent of the 73 million tons currently emitted at low altitudes.85 We won’t be significantly adding to the sulfur burden of the atmosphere through this program. And remember, if we do discover harmful effects, we can always use calcite instead.
Because SO2 does precipitate out of the atmosphere after a couple of years, maintaining a given albedo is a recurring maintenance cost. The upside is that there is a built-in way to dial the albedo down: just stop injecting SO2. This lets us turn the albedo knob in both directions (within limits) without a separate albedo-reducing technology.
Stratospheric aerosol injection is only one form of SRM. For instance, it has been proposed that we could make clouds more reflective by spraying them with seawater from boats.86 Crazier ideas include deploying an enormous sunshade at the L1 Lagrange point in between the Earth and the Sun.87
SRM and other forms of “geoengineering” are controversial, in some circles even taboo.88 One argument is that if we became dependent on SRM, and it ever failed, we’d be in trouble—a scenario known as “termination shock.” But the same argument could be applied to the power grid, our water and sewage infrastructure, our agricultural systems, the global supply chain, and pretty much every other system that constitutes industrial civilization. It is not an argument against SRM, but for robust, reliable SRM. Experience shows that we can make such systems reliable, and that we are better off for it. And the aerosol injection system described above seems like it would be more robust than some other key pieces of infrastructure: it would be less subject to cascading failures than the power grid, and it would have fewer bottlenecks than the global supply chain.
Another argument is “moral hazard”: if SRM works, then it might make people complacent and reduce incentives to decarbonize.89 This argument strongly resonates with a certain framing of climate change: as a morality play, in which humanity has committed the sin of industrialization, we’re now suffering divine punishment for it, and we must repent through degrowth. This frame makes people suspicious of any solution that sounds too easy, cheap, or convenient, which gets derided as a “techno-fix.”90 (Indeed, solutions to climate change seem to be socially acceptable in exact proportion to how expensive they are, a backwards standard.)
If we drop the anti-human moral frame, we can view climate change as a problem of engineering and economics, one that deserves answers based not on moralizing about approaches but on cost-benefit tradeoffs. From that frame, we can see that we should attack the problem from every promising angle, and not taboo any potential piece of the solution.
This is especially true when creating a control system: more levers are better, particularly when they operate at different timescales. And it’s even more clear if we frame the goal as climate safety: safety is almost always achieved through defense in depth, that is, the layering of multiple techniques for redundancy, rather than reliance on any one. Objecting to SRM on moral hazard grounds is like saying that cars shouldn’t have seat belts, because it will reduce the incentive to drive carefully. (Even if this is true, it’s a bad argument against seat belts.)
The conventional moral framing of climate change is actually backwards. Instead of valuing austerity, we should value economic production and its contribution to human well-being. Instead of making a virtue of self-denial, we should make a virtue of human agency and the mastery of nature. In this frame, SRM is just one tool in our toolbox: all tools are good, and a bigger toolbox is better.
One essay can’t do justice to this topic; I have sketched above only the barest outline of a solution. To do so, I have oversimplified the system to a few basic flows. I have mostly ignored methane and lesser GHGs. But from this rough sketch we can envision a path to obtaining mastery over the climate within decades, via a system that would cost less than $1T/yr to operate, and which would avoid trillions or tens of trillions of dollars every year in economic damages.
It’s possible that some of the techniques mentioned above will fail to be effective, economical, or scalable. But there are enough different paths to success that I’m confident we can make one of them work—given sufficient human ambition, dedication, and problem-solving.
If we can do this, then we will not only have prevented needless deaths, and preserved our investments in agriculture and in coastal real estate. We will have achieved the first planetary-scale control of nature—intervening not just in a local region or even a single water system, but in the climate as a whole. This would be a massive step forward for human agency.
- “Only 11 Years Left to Prevent Irreversible Damage”, Piper, “Can We Save the Planet by Shrinking the Economy?”
- Pasquini et al, “Why Some Americans Do Not See Urgency on Climate Change.”
- I use “climate” here in the extended usage, common today, that includes effects on non-climate systems such as ocean acidity.
- IPCC, “Key Risks Across Sectors and Regions.”
- IPCC “Current Status and Trends,” 42–43.
- McPhee, “Atchafalaya” in The Control of Nature.
- Calculations made by ChatGPT 4.5: https://chatgpt.com/share/67f3f220-be2c-800f-b2f6-170c47b3c434.
- IPCC, “The Physical Science Basis,” 181.
- Ritchie et al, “Greenhouse Gas Emissions.”
- IPCC, “The Physical Science Basis,” 471.
- Ritchie, “Where Do Global Greenhouse Gas Emissions Come From?”
- Ritchie and Rosado, “Energy Mix.”
- Center for Climate and Energy Solutions, “Carbon Capture.”
- Baylin-Stern and Berghout, “Is Carbon Capture Too Expensive?”
- Based on average emissions of 823 lbs/MWh = 0.374 kg/kWh of CO2: “Greenhouse Gas Equivalencies Calculator.”
- Average electricity price in the US is about $0.13/kWh: “Electricity Monthly Update.”
- Credit to Alex Epstein for this framing, e.g., in The Moral Case for Fossil Fuels, Chapter 2.
- Unless otherwise cited, all figures are from Sassoon, et al, “Quantifying the Flow of Exergy and Carbon.” The estimates are explained in Hermann, “Quantifying Global Exergy Resources.” The division into stocks and flows here is a judgment call. For sources that have both, I made the call based on the ratio between them, that is, the replenishment time. For instance, there is a stock of about 950 TWy of biomass energy in existing plant growth, but since that’s only about 10x the annual regrowth, it makes more sense to think of it as a flow. Geothermal energy has a comparatively enormous stock of 475 million TWy in the crust, so even though it gets renewed at 32 TW of heat transfer from the mantle—or 1.5x world energy usage today—it makes more sense to think of it as a (practically inexhaustible) stock.
- See energy balance diagram above.
- Ayres and Zamora, “Renewable Power Generation Costs in 2023,” 81–88. The price of solar installations varies by region; it is just over $1/W in the US and less in most other countries. “Electricity cost” refers to the levelized cost of electricity (LCOE), which includes both operating costs and costs to finance the up-front capital investment. Your electricity bill is higher than this because of additional costs, such as the power grid itself.
- OWID, “Solar Power Generation.”
- EIA, “Southwestern States Have Better Solar Resources.” Cembalest, “Heliocentrism.”
- Potter, “Understanding Solar Energy.”
- Apt et al, “Power Play.”
- Ayres and Zamora, “Renewable Power Generation Costs in 2023.”
- For a model of using intermittent solar for local industrial applications, see Handmer, “Solar and Batteries for Generic Use Cases.”
- Lazard, “Levelized Cost of Energy,” 9.
- Touran, “Computing the Energy Density of Nuclear Fuel.”
- Lazard, “Levelized Cost of Energy,” 32.
- Lazard, “Levelized Cost of Energy,” 32–33.
- Nordhaus et al, “How to Make Nuclear Cheap.”
- Lovering et al, “Historical Construction Costs of Global Nuclear Power Reactors”, Dumitriu and Hopkinson, “Infrastructure Costs: Nuclear Edition.”
- Crawford, “Why Has Nuclear Power Been a Flop?”, “Episode 322: Jason Crawford.”
- “Helion Announces First Fusion Purchase with Microsoft”, “World’s First Commercial Fusion Power Plant.”
- “Helion FAQ.”
- Thanks to Eli Dourado, “The State of Next-Generation Geothermal” for bringing this topic to my attention.
- Lazard, “Levelized Cost of Energy,” 9.
- “Fervo Energy Breaks Ground.”
- OWID, “Electricity as a Share of Primary Energy.”
- Based on numbers from “Fast Facts on Transportation Greenhouse Gas Emissions.”
- Mukhopadhaya, “What to Expect When Expecting Electric Airplanes.”
- EIA, “Natural Gas.”
- “The Terraformer Mark One.” Based on 400 million units and 2 million cf/unit/yr, and using a figure of 0.2931 kWh/cf for gas, I calculate an energy production of 26.75 TW.
- “Valar Atomics Comes Out of Stealth.” Long-term targets for fuel prices come from Valar CEO Isaiah Taylor, private correspondence.
- 6,622 TWh of global electricity from natural gas in 2023, or about three-quarters of a terawatt: Ritchie and Rosada, “Electricity Mix.”
- Tomas Pueyo said this before me: “We Can Already Stop Climate Change If We Want To.”
- Bloomberg, “New Energy Outlook 2024” estimates $215 trillion; McKinsey, “The Net-Zero Transition” estimates $275 trillion.
- Allain, “How Much Energy Would it Take to Pull Carbon Dioxide Out of the Air?” gives the theoretical minimum based on thermodynamics as 652,000 J/kg. Hovorka and Keleman, “The Building Blocks of Direct Air Capture” says that with current technology capturing 1 MtCO2/yr requires 180–500 MW. I converted all figures to kWh/t.
- Ozkan et al, “Electrochemical Direct Air Capture.”
- Lebling et al, “6 Things to Know About Direct Air Capture”; Sanz-Pérez et al, “Direct Capture of CO2 from Ambient Air.”
- Pan et al, “A Large and Persistent Carbon Sink in the World’s Forests.”
- Bernal et al, “Global Carbon Dioxide Removal Rates.”
- Ritchie, “Forest Area.”
- Terraformation, “Financing Forests” report, available at “Turning Dollars into Trees.”
- Published sources vary widely; Terraformation CEO Yishan Wong, in private correspondence, cited 128,000 liters/day for 35 acres.
- Global fresh water use was 3.99 trillion m3 in 2014: OWID, “Global Freshwater Use Over the Long Run.”
- Desalination requires 2.5–3.5 kWh/m3 of water: Ritchie, “How Much Energy Does Desalination Use?”
- Terraformation, “Financing Forests” report (available at “Turning Dollars into Trees”) mentions $5/1000 gallons, about $1.32/m3. Bouma et al, “Water for a Warming Climate,” gets less than $1 by repurposing the Diablo Canyon nuclear plant for desalination. Abdulrahim and Ahmed, “Levelized Cost Analysis for Desalination,” gets just over $1 using solar power.
- Yishan Wong, private correspondence. Wong reported that equipment taking up less than an acre, including solar panels and water tanks, could irrigate 35 acres of forest, based on his experiments at Terraformation’s North Kohala station in Hawaii.
- Terraformation, “Financing Forests” report, available at “Turning Dollars into Trees.”
- Lindsey and Scott, “What are Phytoplankton?”
- Bristow et al, “Nutrients that Limit Growth in the Ocean.”
- Markels Jr. and Barber, “Sequestration of Carbon Dioxide by Ocean Fertilization.” Emerson et al, “A Cost Model for Ocean Iron Fertilization” provides a detailed set of estimates including verification, which could significantly increase the price, and gets an intermediate-case number using aerial delivery of $83/t of C, which is ~$23/t of CO2.
- “Ocean Phytoplankton.”
- Marblestone, “Climate Technology Primer,” 2.10.
- See “Biodiversity and Climate Change” and “Ocean Fertilization Under the LC/LP”, or for a primer on both see Currie, “A Brief Primer on Ocean Fertilization.”
- Marblestone, “Climate Technology Primer,” 2.7.
- Marblestone, “Climate Technology Primer,” 2.10.
- Hovorka and Keleman, “Building Blocks of Direct Air Capture,” 2.1.
- See carbon balance diagram above, which gives the figure as 0.3 Gt of carbon.
- Hovorka and Keleman, “Building Blocks of Direct Air Capture,” 2.1.
- The largest estimate I have seen for remaining fossil fuels comes from Sassoon, et al, “Quantifying the Flow of Exergy and Carbon”: 8,000 Gt of carbon from coal, 2,300 from oil, and 830 from gas. That much carbon would create about 40,800 Gt of CO2.
- Nilsen, “Olivine Weathering.”
- “Cell Free Systems.”
- As per the carbon balance figures above, the atmosphere contains some 840 Gt of carbon, which means over 3,000 Gt of CO2.
- Marblestone, “Climate Technology Primer,” 3.2.
- See energy balance diagram above.
- Credit to Pueyo, “How You Can Easily Delay Climate Change Today,” for bringing this to my attention.
- The calculations are given and key inputs cited in Iseman, “Calculating Cooling.” The figure there is given as -0.31 W/m2 radiative forcing per Tg SO2/year.
- NASA, “Global Effects of Mount Pinatubo.” Various sources cite numbers from 15 to 20 million tons of SO2.
- “Cooling Credits.”
- Handmer, “We Should Not Let the Earth Overheat!” Andrew Song, co-founder of Make Sunsets, confirmed in private correspondence that this estimate is realistic long-term.
- “What is Acid Rain?”
- Crutzen, “Albedo Enhancement by Stratospheric Sulfur Injections.”
- OWID, “Global Sulphur Dioxide Emissions by World Region.”
- Marblestone, “Climate Technology Primer,” 3.3.
- Angel, “Feasibility of Cooling the Earth with a Cloud of Small Spacecraft.”
- Carlson and Church, “The Risks of Geoengineering.”, Robock, “20 Reasons Why Geoengineering May be a Bad Idea.”
- McLaren, “Mitigation Deterrence and the Moral Hazard of Solar Radiation Management.”
- “Solar Radiation Management: Who Should Control the Weather?”